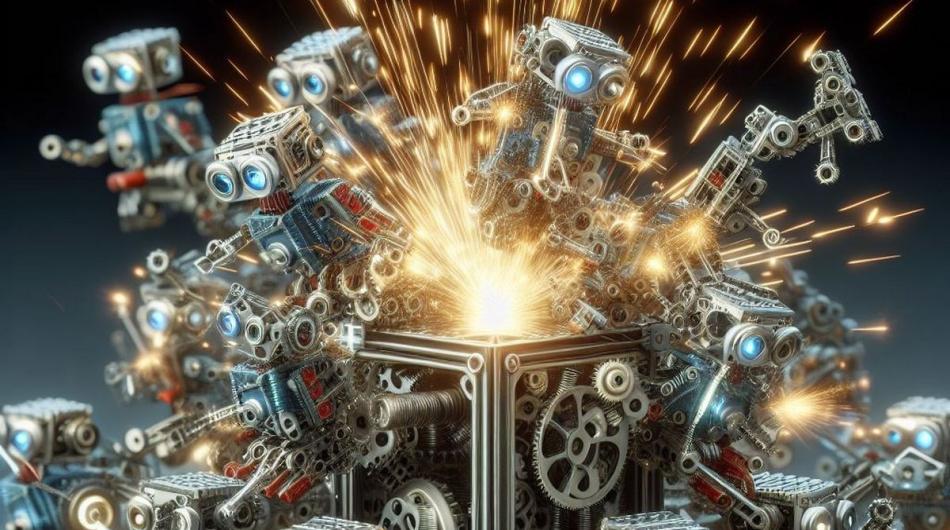
Self-assembling materials are a fascinating class of substances that can spontaneously organize themselves into ordered structures without external intervention. This process, known as self-assembly, is a fundamental phenomenon in nature and is responsible for the formation of complex systems ranging from the molecular structure of DNA to the intricate patterns of seashells. In recent years, scientists and engineers have sought to harness the power of self-assembly to create novel materials with unique properties and functionalities.
Principles of Self-Assembly
Definition and Overview
Self-assembly refers to the process by which individual components or building blocks spontaneously organize themselves into ordered structures without human intervention. This process is driven by the interactions between the components, which can be governed by various forces such as hydrogen bonding, electrostatic interactions, van der Waals forces, and hydrophobic effects. Self-assembly can occur at different length scales, from the molecular level to the macroscopic scale, and can involve a wide range of materials, including polymers, nanoparticles, and biomolecules.
The concept of self-assembly is inspired by nature, where many complex structures and functions emerge from the spontaneous organization of simple building blocks. For example, the formation of cell membranes, the folding of proteins, and the assembly of viruses are all examples of self-assembly in biological systems. By understanding and mimicking these natural processes, scientists aim to create synthetic materials with similar properties and capabilities.
Thermodynamic and Kinetic Aspects
The process of self-assembly is governed by the interplay between thermodynamics and kinetics. From a thermodynamic perspective, self-assembly occurs when the system minimizes its free energy by adopting an ordered configuration. This means that the interactions between the components must be favorable enough to overcome the entropic cost of organizing into a structured arrangement.
The kinetics of self-assembly, on the other hand, determine the pathway and rate at which the components assemble into the final structure. Kinetic factors such as the diffusion rate of the components, the presence of energy barriers, and the reversibility of the interactions can influence the assembly process and the quality of the resulting structure.
To achieve successful self-assembly, it is essential to carefully design the building blocks and the environment in which they interact. This involves selecting components with complementary interactions, controlling the stoichiometry and concentration of the components, and tuning the external conditions such as temperature, pH, and solvent quality.
Types of Self-Assembly
Self-assembly can be classified into different categories based on the nature of the interactions and the resulting structures. Some common types of self-assembly include:
- Molecular Self-Assembly: This involves the spontaneous organization of molecules into ordered structures such as crystals, liquid crystals, or self-assembled monolayers. Examples include the formation of lipid bilayers in cell membranes and the assembly of block copolymers into micelles or vesicles.
- Supramolecular Self-Assembly: This refers to the assembly of supramolecular structures from smaller molecular components held together by non-covalent interactions such as hydrogen bonding, π-π stacking, or metal-ligand coordination. Examples include the formation of host-guest complexes, rotaxanes, and catenanes.
- Colloidal Self-Assembly: This involves the organization of colloidal particles, typically in the size range of nanometers to micrometers, into ordered arrays or structures. Examples include the assembly of nanoparticles into superlattices, the formation of colloidal crystals, and the self-assembly of patchy particles.
- Biomolecular Self-Assembly: This refers to the self-assembly of biological molecules such as proteins, nucleic acids, and lipids into functional structures. Examples include the folding of proteins into their native conformations, the assembly of virus capsids, and the formation of DNA origami structures.
Applications of Self-Assembling Materials
Self-assembling materials have attracted significant interest due to their potential applications in various fields, ranging from materials science and nanotechnology to medicine and biotechnology. Some of the most promising applications of self-assembling materials include:
Nanofabrication and Electronics
Self-assembly provides a powerful tool for creating ordered nanostructures and devices with precise control over their size, shape, and composition. By designing building blocks with specific interactions and geometries, it is possible to direct their self-assembly into complex architectures such as nanowires, nanorods, and nanotubes. These self-assembled nanostructures can be used as templates for the fabrication of electronic devices, photonic crystals, and metamaterials with unique optical and electronic properties.
One example of self-assembly in nanofabrication is the use of block copolymers, which are polymers consisting of two or more chemically distinct blocks. By controlling the composition and architecture of the block copolymers, it is possible to direct their self-assembly into periodic nanostructures such as lamellar, cylindrical, or gyroidal phases. These self-assembled block copolymer nanostructures can be used as templates for the fabrication of nanoporous membranes, nanoelectronic devices, and bit-patterned media for high-density data storage.
Biomaterials and Tissue Engineering
Self-assembling materials have significant potential in the field of biomaterials and tissue engineering, where they can be used to create scaffolds and matrices that mimic the natural extracellular environment. By designing building blocks with biocompatible and biodegradable properties, it is possible to create self-assembling materials that can support cell adhesion, proliferation, and differentiation.
One example of self-assembling biomaterials is the use of peptide amphiphiles, which are molecules consisting of a hydrophobic alkyl tail and a hydrophilic peptide head group. By controlling the sequence and structure of the peptide head group, it is possible to design peptide amphiphiles that self-assemble into nanofibers or hydrogels with specific biological functions. These self-assembling peptide materials have been used as scaffolds for tissue engineering, drug delivery systems, and biosensors.
Another example is the use of DNA origami, which involves the self-assembly of DNA molecules into precise two- and three-dimensional shapes. By designing DNA sequences with complementary base pairing, it is possible to create complex DNA nanostructures such as boxes, cages, and tubes. These DNA origami structures have been used as drug-delivery vehicles, molecular machines, and templates for the assembly of proteins and nanoparticles.
Smart Materials and Sensors
Self-assembling materials can also be used to create smart materials and sensors that respond to external stimuli such as temperature, pH, light, or magnetic fields. By incorporating functional groups or nanoparticles into the self-assembling building blocks, it is possible to create materials with stimuli-responsive properties such as color change, shape change, or conductivity change.
One example of self-assembling smart materials is the use of liquid crystalline polymers, which are polymers that exhibit liquid crystalline behavior. By designing liquid crystalline polymers with specific mesogenic units and stimuli-responsive groups, it is possible to create materials that change their optical, mechanical, or electrical properties in response to external stimuli. These self-assembling liquid crystalline polymers have been used as sensors, actuators, and displays.
Another example is the use of self-assembling metal-organic frameworks (MOFs), which are porous crystalline materials composed of metal ions or clusters coordinated to organic ligands. By selecting metal ions and ligands with specific properties, it is possible to create MOFs with tailored pore sizes, surface areas, and functionalities. These self-assembling MOFs have been used as gas storage materials, catalysts, and chemical sensors.
Energy and Catalysis
Self-assembling materials have also found applications in the field of energy and catalysis, where they can be used to create functional materials for energy conversion and storage, as well as catalytic reactions. By designing building blocks with specific electronic and structural properties, it is possible to create self-assembling materials with enhanced charge transport, light harvesting, or catalytic activity.
One example of self-assembling materials for energy applications is the use of self-assembling organic semiconductors, which are materials that exhibit semiconducting properties due to their conjugated molecular structure. By controlling the self-assembly of organic semiconductors into ordered nanostructures such as nanowires or nanofibers, it is possible to create materials with improved charge carrier mobility and conductivity. These self-assembling organic semiconductors have been used in organic photovoltaics, light-emitting diodes, and field-effect transistors.
Another example is the use of self-assembling nanoparticle catalysts, which are nanoparticles that exhibit catalytic activity due to their high surface area and specific surface chemistry. By controlling the self-assembly of nanoparticles into ordered structures such as superlattices or core-shell particles, it is possible to create catalysts with enhanced activity, selectivity, and stability. These self-assembling nanoparticle catalysts have been used in various catalytic reactions such as hydrogenation, oxidation, and coupling reactions.
Challenges and Future Prospects
Despite the significant progress made in the field of self-assembling materials, there are still several challenges and opportunities for future research and development.
Challenges
One of the main challenges in self-assembling materials is the precise control over the assembly process and the resulting structures. While self-assembly is a spontaneous process, it is often sensitive to small changes in the environment or the building blocks, which can lead to defects or irregularities in the final structure. Therefore, it is important to develop robust and reproducible self-assembly strategies that can tolerate variations in the experimental conditions.
Another challenge is the scalability and manufacturability of self-assembling materials. While self-assembly is a powerful tool for creating complex nanostructures, it is often difficult to scale up the production of these materials to industrially relevant quantities. Therefore, it is important to develop scalable and cost-effective manufacturing processes that can translate the self-assembly principles from the laboratory to the factory.
A third challenge is the integration of self-assembling materials with other materials and devices. While self-assembling materials can exhibit unique properties and functionalities, they often need to be integrated with other materials or devices to create practical applications. Therefore, it is important to develop compatible and reliable interfaces between self-assembling materials and other components, as well as to ensure the long-term stability and performance of the integrated systems.
Future Prospects
Despite these challenges, the field of self-assembling materials offers exciting opportunities for future research and innovation. One of the key areas of focus is the development of multi-functional and responsive self-assembling materials that can perform multiple tasks or adapt to changing environments. By incorporating different functional groups or stimuli-responsive moieties into the building blocks, it is possible to create materials that can sense, actuate, or communicate in response to external signals.
Another area of interest is the integration of self-assembling materials with living systems, such as cells or tissues. By designing self-assembling materials that can interact with biological molecules or cells, it is possible to create hybrid materials that can interface with living organisms for applications such as drug delivery, tissue engineering, or biosensing. This requires a deep understanding of the biological and chemical principles governing the interactions between self-assembling materials and living systems.
A third area of opportunity is the development of computational tools and methods for designing and predicting the self-assembly of materials. With the increasing complexity and diversity of self-assembling building blocks, it is becoming increasingly important to use computational modeling and simulation to guide the design and optimization of self-assembling materials. This requires the development of accurate and efficient computational methods that can capture the thermodynamic and kinetic aspects of self-assembly, as well as the integration of these methods with experimental characterization techniques.
Finally, the field of self-assembling materials offers opportunities for interdisciplinary collaboration and innovation. Self-assembly is a fundamental principle that cuts across different fields, from materials science and chemistry to biology and physics. Therefore, it is important to foster collaboration and knowledge exchange between different disciplines to accelerate the development and application of self-assembling materials. This requires the establishment of interdisciplinary research centers, training programs, and funding mechanisms that can support the growth and sustainability of this field.
Conclusion
Self-assembling materials are a fascinating and promising class of materials that can spontaneously organize into ordered structures with unique properties and functionalities. By harnessing the principles of self-assembly, it is possible to create complex and diverse materials that can rival the sophistication and efficiency of natural systems. Self-assembling materials have already found applications in various fields, from nanofabrication and biomaterials to smart materials and energy, and are expected to play an increasingly important role in the development of future technologies.
However, the field of self-assembling materials also faces several challenges and opportunities that require further research and innovation. These include the precise control over the assembly process, the scalability and manufacturability of self-assembling materials, the integration with other materials and devices, and the development of multi-functional and responsive materials. To address these challenges and realize the full potential of self-assembling materials, it is important to foster interdisciplinary collaboration, develop new computational tools and methods, and establish supportive research and funding environments.
In conclusion, self-assembling materials represent a fascinating and rapidly evolving field that offers exciting opportunities for scientific discovery and technological innovation. With the continued advancement of our understanding and control over self-assembly, it is possible to create materials with unprecedented properties and functionalities that can transform various aspects of our lives, from healthcare and energy to electronics and sustainability. As such, the field of self-assembling materials is poised to play a crucial role in shaping the future of materials science and technology, and will undoubtedly remain an active and vibrant area of research and development in the years to come.