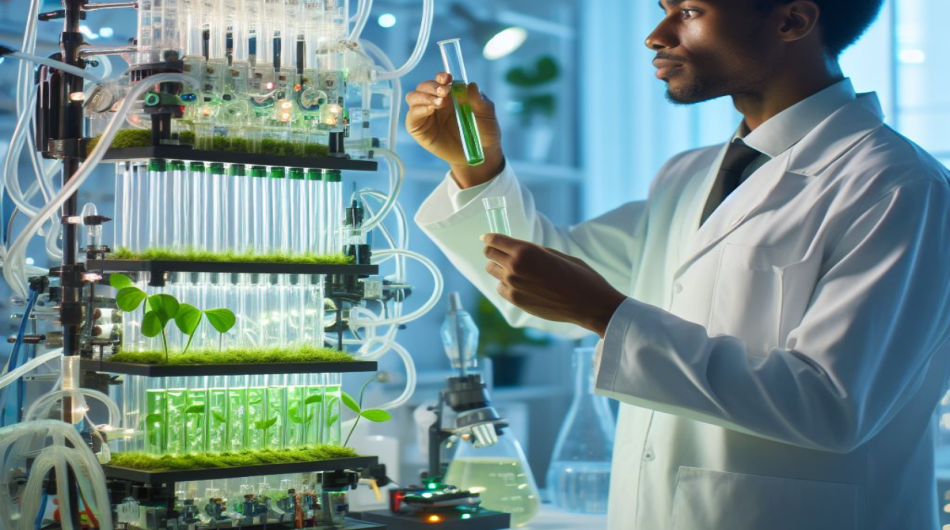
Artificial photosynthesis is a rapidly evolving field that aims to mimic and improve upon the natural process of photosynthesis, which harnesses solar energy to convert water and carbon dioxide into carbohydrates and oxygen. By developing efficient and scalable artificial photosynthetic systems, researchers hope to create sustainable solutions for renewable energy production, carbon dioxide reduction, and the synthesis of valuable chemicals and fuels. This article provides a comprehensive overview of artificial photosynthesis, including its fundamental principles, key components, current research progress, challenges, and future prospects.
Fundamental Principles of Artificial Photosynthesis
Artificial photosynthesis draws inspiration from the natural process of photosynthesis, which has evolved over billions of years to optimize the capture and conversion of solar energy into chemical energy. In natural photosynthesis, light-harvesting complexes in plants and other photosynthetic organisms absorb sunlight and transfer the energy to reaction centers, where it drives the oxidation of water and the reduction of carbon dioxide to produce carbohydrates and oxygen.
Artificial photosynthetic systems aim to replicate this process using synthetic components, such as semiconductors, catalysts, and membranes. The basic principles of artificial photosynthesis involve:
-
Light absorption: Capturing solar energy using light-harvesting materials, such as semiconductors or dye molecules.
-
Charge separation: Separating the photogenerated electrons and holes to prevent their recombination and enable their use in redox reactions.
-
Water oxidation: Using the photogenerated holes to oxidize water, producing protons and oxygen.
-
CO2 reduction or fuel production: Using the photogenerated electrons to reduce carbon dioxide or other substrates, producing valuable chemicals or fuels.
By optimizing each of these steps and integrating them into a functional system, artificial photosynthesis has the potential to provide a sustainable and scalable solution for converting solar energy into storable and transportable chemical energy.
Advantages of Artificial Photosynthesis
Artificial photosynthesis offers several key advantages over natural photosynthesis and other renewable energy technologies:
-
Higher efficiency: Artificial photosynthetic systems can be designed to absorb a wider range of the solar spectrum and convert energy more efficiently than natural photosynthesis, which has a theoretical maximum efficiency of around 11% for crop plants.
-
Controllable products: By selecting appropriate catalysts and reaction conditions, artificial photosynthesis can be tuned to produce specific chemicals or fuels, such as hydrogen, methanol, or ethylene, depending on the desired application.
-
Scalability: Artificial photosynthetic systems can be designed as modular and scalable devices, enabling their deployment in a wide range of settings, from small-scale distributed systems to large-scale centralized plants.
-
CO2 reduction: By using carbon dioxide as a feedstock, artificial photosynthesis can contribute to the mitigation of greenhouse gas emissions and the creation of a closed-loop carbon cycle.
Challenges in Artificial Photosynthesis
Despite its potential benefits, artificial photosynthesis faces several challenges that must be addressed to achieve practical and economical implementation:
-
Materials development: Identifying and optimizing materials that can efficiently absorb light, separate charges, and catalyze the desired redox reactions is a key challenge. These materials must be stable, abundant, and cost-effective to enable large-scale deployment.
-
System integration: Integrating the various components of an artificial photosynthetic system, such as light absorbers, catalysts, and membranes, into a functional and efficient device requires careful design and optimization.
-
Scalability and durability: Developing artificial photosynthetic systems that can operate efficiently and reliably over extended periods and under real-world conditions is essential for practical applications.
-
Cost and economic viability: Reducing the cost of materials, fabrication, and operation to make artificial photosynthesis economically competitive with other renewable energy technologies is a significant challenge.
Researchers are actively working to address these challenges through a combination of fundamental studies, materials discovery, device engineering, and techno-economic analysis.
Key Components of Artificial Photosynthetic Systems
Artificial photosynthetic systems typically consist of several key components that work together to absorb light, separate charges, and drive the desired redox reactions. These components include:
Light Absorbers
Light absorbers are materials that capture solar energy and generate excited electrons and holes. The most common types of light absorbers used in artificial photosynthesis are semiconductors, which have a band gap that allows them to absorb photons of specific energies. Some examples of semiconductor light absorbers include:
-
Silicon: Silicon is the most widely used semiconductor in photovoltaic devices and has also been employed in artificial photosynthetic systems due to its abundance, stability, and well-established fabrication methods.
-
Metal oxides: Metal oxides, such as titanium dioxide (TiO2), tungsten trioxide (WO3), and hematite (α-Fe2O3), have been extensively studied as light absorbers in artificial photosynthesis due to their stability, low cost, and suitable band gaps.
-
III-V semiconductors: Semiconductors composed of elements from groups III and V of the periodic table, such as gallium arsenide (GaAs) and indium phosphide (InP), have high absorption coefficients and tunable band gaps, making them attractive for artificial photosynthesis.
In addition to semiconductors, other materials, such as organic dyes and quantum dots, have also been explored as light absorbers in artificial photosynthetic systems.
Catalysts
Catalysts are materials that facilitate the water oxidation and CO2 reduction reactions in artificial photosynthesis by lowering the activation energy and providing reaction sites. The choice of catalyst is critical for determining the efficiency, selectivity, and stability of the system. Some common types of catalysts used in artificial photosynthesis include:
-
Metal complexes: Molecular metal complexes, such as those based on ruthenium, iridium, and cobalt, have been widely studied as catalysts for water oxidation and CO2 reduction due to their high activity and selectivity.
-
Metal oxides: Metal oxides, such as iridium oxide (IrO2) and cobalt oxide (CoOx), are attractive catalysts for water oxidation due to their stability and high activity.
-
Enzymes and bioinspired catalysts: Natural enzymes, such as photosystem II and formate dehydrogenase, and their synthetic analogs have been explored as catalysts for artificial photosynthesis due to their high efficiency and selectivity.
-
Nanomaterials: Nanostructured materials, such as nanoparticles, nanowires, and nanosheets, have high surface areas and unique electronic properties that can enhance catalytic activity and stability.
Researchers are continually developing and optimizing catalysts to improve the efficiency and selectivity of artificial photosynthetic systems while reducing their cost and complexity.
Membranes and Separators
Membranes and separators are essential components in artificial photosynthetic systems, as they allow the selective transport of reactants and products while preventing the mixing of incompatible species. The choice of membrane or separator depends on the specific requirements of the system, such as pH, temperature, and chemical compatibility. Some common types of membranes and separators used in artificial photosynthesis include:
-
Proton exchange membranes (PEMs): PEMs, such as Nafion, are polymer membranes that allow the selective transport of protons while blocking the passage of other species. They are widely used in fuel cells and have also been employed in artificial photosynthetic systems for separating the water oxidation and CO2 reduction compartments.
-
Ion exchange membranes: Ion exchange membranes, such as anion exchange membranes (AEMs) and cation exchange membranes (CEMs), allow the selective transport of specific ions based on their charge. They can be used to maintain charge balance and prevent the crossover of reactants and products in artificial photosynthetic systems.
-
Porous separators: Porous separators, such as glass frits and ceramics, allow the passage of small molecules while blocking larger species. They can be used to separate the anodic and cathodic compartments in artificial photosynthetic systems while allowing the transport of protons and other necessary species.
The development of advanced membranes and separators with improved selectivity, stability, and conductivity is an active area of research in artificial photosynthesis.
Current Research Progress and Achievements
Research in artificial photosynthesis has made significant progress in recent years, with advances in materials development, device design, and system integration. Some notable achievements include:
Improved Light Absorption and Charge Separation
Researchers have developed new light-absorbing materials and device architectures that can capture a wider range of the solar spectrum and efficiently separate photogenerated charges. For example:
-
Tandem devices: By combining multiple light absorbers with complementary band gaps, tandem devices can harvest a larger portion of the solar spectrum and achieve higher overall efficiencies than single-absorber devices.
-
Nanostructured materials: Nanostructured light absorbers, such as nanowires and nanosheets, can enhance light absorption and charge separation due to their high surface area and reduced charge transport distances.
-
Plasmonic materials: Incorporating plasmonic nanostructures, such as metal nanoparticles, into light absorbers can increase light absorption and charge separation through localized surface plasmon resonance effects.
Advanced Catalysts and Reaction Systems
Researchers have developed new catalysts and reaction systems that can efficiently drive the water oxidation and CO2 reduction reactions in artificial photosynthesis. For example:
-
Molecular catalysts: Molecular catalysts based on abundant metals, such as iron and cobalt, have been developed as alternatives to rare and expensive noble metal catalysts. These catalysts can achieve high activities and selectivities for water oxidation and CO2 reduction.
-
Nanostructured catalysts: Nanostructured catalysts, such as nanoparticles and nanosheets, can provide high surface areas and active sites for catalysis while improving stability and charge transfer.
-
Biohybrid systems: Integrating natural enzymes or their synthetic analogs with artificial photosynthetic systems can enhance the efficiency and selectivity of the catalytic reactions while providing a route to more sustainable and biocompatible systems.
Integrated Devices and Prototypes
Researchers have developed integrated devices and prototypes that demonstrate the feasibility and potential of artificial photosynthesis for renewable energy production and CO2 reduction. For example:
-
Wireless devices: Wireless artificial photosynthetic devices that can operate autonomously under natural sunlight have been developed, demonstrating the potential for decentralized and off-grid applications.
-
Solar-driven CO2 reduction: Integrated systems that can efficiently reduce CO2 to valuable chemicals and fuels, such as carbon monoxide, formic acid, and methanol, using solar energy have been demonstrated at various scales.
-
Solar hydrogen production: Artificial photosynthetic devices that can split water into hydrogen and oxygen using solar energy have been developed, providing a route to clean and sustainable hydrogen production.
These achievements highlight the progress and potential of artificial photosynthesis, but there is still much work to be done to further improve efficiency, stability, scalability, and economic viability.
Challenges and Future Directions
Despite the significant progress made in artificial photosynthesis research, there are still several challenges and opportunities for future development:
Materials Discovery and Optimization
Identifying and optimizing materials that can efficiently absorb light, separate charges, and catalyze the desired reactions is a key challenge in artificial photosynthesis. Future research directions in this area include:
-
High-throughput screening: Using computational and experimental high-throughput screening methods to rapidly identify and optimize new materials with desired properties for artificial photosynthesis.
-
Earth-abundant materials: Developing artificial photosynthetic systems based on earth-abundant and low-cost materials to improve scalability and economic viability.
-
Bioinspired materials: Drawing inspiration from natural photosynthetic systems to design more efficient and selective materials for artificial photosynthesis.
System Integration and Optimization
Integrating the various components of an artificial photosynthetic system into a functional and efficient device requires careful design and optimization. Future research directions in this area include:
-
Multiphysics modeling: Using advanced multiphysics modeling tools to simulate and optimize the performance of artificial photosynthetic systems under realistic conditions.
-
Microfluidic devices: Developing microfluidic artificial photosynthetic devices that can precisely control the transport and reaction of species while minimizing losses and improving efficiency.
-
Tandem and hybrid systems: Integrating artificial photosynthetic systems with other renewable energy technologies, such as photovoltaics or electrolyzers, to create tandem or hybrid devices with improved overall efficiency and functionality.
Scalability and Techno-Economic Analysis
Demonstrating the scalability and economic viability of artificial photosynthetic systems is essential for their practical implementation. Future research directions in this area include:
-
Pilot-scale demonstrations: Conducting pilot-scale demonstrations of artificial photosynthetic systems under real-world conditions to validate their performance and identify potential challenges and bottlenecks.
-
Techno-economic analysis: Performing detailed techno-economic analyses to assess the feasibility and competitiveness of artificial photosynthetic systems compared to other renewable energy and CO2 reduction technologies.
-
Life cycle assessment: Conducting life cycle assessments to evaluate the environmental impacts and sustainability of artificial photosynthetic systems throughout their entire life cycle, from materials extraction to end-of-life disposal.
Societal and Policy Implications
The development and deployment of artificial photosynthetic systems have significant societal and policy implications that must be considered. Future research directions in this area include:
-
Public engagement and education: Engaging with the public and stakeholders to raise awareness and understanding of artificial photosynthesis and its potential benefits and risks.
-
Policy and regulatory frameworks: Developing appropriate policy and regulatory frameworks to support the responsible development and deployment of artificial photosynthetic systems, considering factors such as safety, environmental impact, and social equity.
-
Interdisciplinary collaboration: Fostering interdisciplinary collaboration among scientists, engineers, social scientists, policymakers, and industry partners to address the complex challenges and opportunities of artificial photosynthesis.
By addressing these challenges and opportunities, artificial photosynthesis has the potential to become a transformative technology for sustainable energy production and CO2 reduction, contributing to the mitigation of climate change and the transition to a more sustainable future.
Conclusion
Artificial photosynthesis is a promising and rapidly evolving field that aims to harness the power of sunlight to produce renewable fuels and chemicals while reducing atmospheric CO2 levels. By mimicking and improving upon the natural process of photosynthesis, artificial photosynthetic systems have the potential to provide a sustainable and scalable solution for meeting the world's growing energy demands while combating climate change.
Recent advancements in materials development, device design, and system integration have demonstrated the feasibility and potential of artificial photosynthesis, but there are still significant challenges to be addressed in terms of efficiency, stability, scalability, and economic viability. Ongoing research efforts in areas such as materials discovery, system optimization, techno-economic analysis, and policy development are critical for realizing the full potential of artificial photosynthesis.
As the world faces the urgent need to transition to a more sustainable and carbon-neutral future, artificial photosynthesis represents a promising pathway for harnessing the abundant and renewable energy of the sun to power our societies and economies. With continued research, innovation, and collaboration, artificial photosynthesis could play a crucial role in mitigating climate change, reducing our dependence on fossil fuels, and creating a more sustainable and resilient world for future generations.