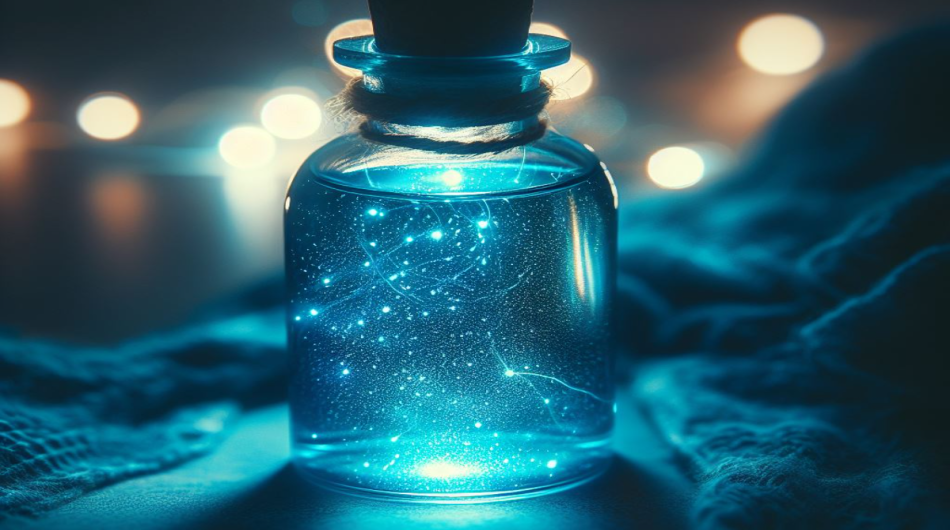
Advanced materials are substances that have been engineered to possess unique properties, structures, or functions that surpass those of conventional materials. These materials are designed to meet specific performance requirements and address the challenges faced by various industries, such as aerospace, automotive, energy, healthcare, and electronics. The development of advanced materials has revolutionized many aspects of modern life and continues to drive innovation and technological progress. In this article, we will explore the different types of advanced materials, their properties, applications, and the challenges and future prospects of this field.
Types of Advanced Materials
Advanced materials can be classified into several categories based on their composition, structure, and properties. Some of the main types of advanced materials include:
Nanomaterials
Nanomaterials are substances that have at least one dimension in the nanoscale range (1-100 nanometers). These materials exhibit unique properties that differ from their bulk counterparts due to their high surface area-to-volume ratio and quantum confinement effects. Examples of nanomaterials include:
-
Carbon nanotubes: Cylindrical structures made of carbon atoms with exceptional mechanical, thermal, and electrical properties
-
Graphene: A two-dimensional form of carbon with high strength, conductivity, and transparency
-
Nanoparticles: Particles with diameters in the nanoscale range, such as gold, silver, and titanium dioxide nanoparticles, which have applications in catalysis, sensing, and medicine
Metamaterials
Metamaterials are artificial materials engineered to have properties that are not found in naturally occurring substances. These materials are designed by arranging conventional materials in specific patterns or structures, resulting in unique electromagnetic, acoustic, or optical properties. Examples of metamaterials include:
-
Negative index materials: Materials that exhibit a negative refractive index, allowing for the creation of "superlenses" and cloaking devices
-
Acoustic metamaterials: Materials that can manipulate sound waves, enabling the development of noise-canceling devices and acoustic cloaking
-
Photonic crystals: Materials with periodic variations in their dielectric properties, allowing for the control of light propagation and the creation of optical filters and waveguides
Smart Materials
Smart materials are substances that can change their properties in response to external stimuli, such as temperature, pressure, electric or magnetic fields, or chemical environment. These materials have the ability to sense, process, and respond to their surroundings, making them suitable for a wide range of applications. Examples of smart materials include:
-
Shape memory alloys: Materials that can return to their pre-deformed shape when heated, such as nickel-titanium alloys (Nitinol) used in medical devices and actuators
-
Piezoelectric materials: Materials that generate an electric charge when subjected to mechanical stress, or vice versa, such as lead zirconate titanate (PZT) used in sensors and energy harvesting devices
-
Magnetorheological fluids: Fluids that can change their viscosity in response to a magnetic field, enabling the development of adaptive dampers and brakes
Biomaterials
Biomaterials are substances designed to interact with biological systems for medical purposes, such as tissue engineering, drug delivery, or implantable devices. These materials are engineered to be biocompatible, biodegradable, or bioactive, depending on their intended application. Examples of biomaterials include:
-
Hydrogels: Polymer networks that can absorb large amounts of water, making them suitable for tissue engineering scaffolds and drug delivery systems
-
Bioceramics: Ceramic materials that are biocompatible and can bond with living tissues, such as hydroxyapatite used in bone implants and dental restorations
-
Biopolymers: Polymers derived from natural sources, such as collagen, chitosan, and cellulose, which have applications in wound healing, tissue regeneration, and drug delivery
Composite Materials
Composite materials are formed by combining two or more distinct materials to achieve properties that are superior to those of the individual components. These materials often consist of a matrix (continuous phase) and reinforcement (dispersed phase), which can be fibers, particles, or flakes. Examples of composite materials include:
-
Fiber-reinforced polymers: Polymers reinforced with high-strength fibers, such as carbon, glass, or aramid, used in aerospace, automotive, and sports equipment applications
-
Metal matrix composites: Metals reinforced with ceramic particles or fibers, such as aluminum-silicon carbide composites used in aerospace and automotive components
-
Ceramic matrix composites: Ceramics reinforced with ceramic fibers or whiskers, such as silicon carbide-silicon carbide composites used in high-temperature applications, such as jet engines and gas turbines
Properties of Advanced Materials
Advanced materials are designed to possess unique properties that enable them to perform specific functions or meet particular performance requirements. Some of the key properties of advanced materials include:
Mechanical Properties
-
High strength-to-weight ratio: Advanced materials, such as carbon fiber composites, can offer high strength while being significantly lighter than traditional materials like steel or aluminum
-
Enhanced toughness: Materials like ceramic matrix composites can withstand high stresses and strains without fracturing, making them suitable for demanding applications
-
Improved wear resistance: Advanced materials, such as diamond-like carbon coatings, can resist wear and tear, extending the lifespan of components and reducing maintenance requirements
Thermal Properties
-
High-temperature stability: Materials like ceramics and superalloys can maintain their properties at elevated temperatures, enabling their use in extreme environments such as jet engines and nuclear reactors
-
Thermal insulation: Advanced insulation materials, such as aerogels and vacuum insulated panels, can provide superior thermal insulation, reducing energy consumption in buildings and appliances
-
Thermal conductivity: Materials like diamond and graphene have exceptionally high thermal conductivity, making them useful for heat dissipation in electronics and other applications
Electrical and Magnetic Properties
-
Superconductivity: Some advanced materials, such as high-temperature superconductors, can conduct electricity with zero resistance, enabling the development of efficient power transmission and storage systems
-
Magnetoresistance: Materials like giant magnetoresistive (GMR) sensors can change their electrical resistance in response to magnetic fields, enabling the creation of high-density data storage devices and sensitive magnetic field detectors
-
Piezoelectricity: Piezoelectric materials can generate an electric charge when subjected to mechanical stress, or vice versa, making them useful for sensors, actuators, and energy harvesting applications
Optical Properties
-
Transparency: Advanced materials like transparent conducting oxides (TCOs) can combine high electrical conductivity with optical transparency, enabling the development of touch screens and solar cells
-
Photochromism: Photochromic materials can change their color or opacity in response to light, enabling the creation of adaptive lenses and smart windows
-
Luminescence: Materials like quantum dots and phosphors can emit light when excited by an external energy source, enabling the development of energy-efficient lighting and display technologies
Biological Properties
-
Biocompatibility: Advanced biomaterials are designed to be compatible with living tissues, minimizing the risk of adverse immune reactions and promoting tissue integration
-
Biodegradability: Some biomaterials are engineered to degrade at a controlled rate within the body, enabling the development of temporary implants and drug delivery systems
-
Bioactivity: Bioactive materials can stimulate specific biological responses, such as promoting bone growth or preventing bacterial adhesion, making them useful for tissue engineering and medical device applications
Applications of Advanced Materials
Advanced materials have found applications in a wide range of industries, driving innovation and enabling the development of new technologies. Some of the key application areas include:
Aerospace and Automotive
-
Lightweight structural components: Advanced materials like carbon fiber composites and titanium alloys are used to create lightweight and strong components for aircraft and vehicles, improving fuel efficiency and performance
-
Thermal protection systems: Ceramic matrix composites and high-temperature alloys are used in the hot sections of jet engines and spacecraft to withstand extreme temperatures and stresses
-
Energy storage: Advanced materials like lithium-ion batteries and supercapacitors are used to store and deliver energy in electric and hybrid vehicles, enabling longer driving ranges and faster charging times
Energy and Environment
-
Solar cells: Advanced materials like perovskites and organic semiconductors are being developed to create more efficient and cost-effective solar cells, enabling the wider adoption of renewable energy
-
Fuel cells: Advanced materials like proton exchange membranes and catalyst materials are used in fuel cells to convert chemical energy directly into electricity, with applications in transportation and stationary power generation
-
Carbon capture and storage: Advanced materials like metal-organic frameworks (MOFs) and zeolites are being investigated for their ability to selectively capture and store carbon dioxide, helping to mitigate climate change
Electronics and Communication
-
Semiconductor devices: Advanced materials like gallium nitride (GaN) and silicon carbide (SiC) are used to create high-performance and energy-efficient power electronics, enabling the development of faster and more compact devices
-
Optical fibers: Advanced materials like low-loss silica and chalcogenide glasses are used to create optical fibers for high-speed data transmission, enabling the expansion of broadband internet and telecommunications networks
-
Flexible electronics: Advanced materials like conductive polymers and graphene are being developed to create flexible and stretchable electronic devices, such as wearable sensors and displays
Healthcare and Medicine
-
Drug delivery: Advanced materials like liposomes and polymeric nanoparticles are used to encapsulate and deliver drugs to specific targets in the body, improving therapeutic efficacy and reducing side effects
-
Tissue engineering: Advanced biomaterials like hydrogels and biodegradable polymers are used to create scaffolds for tissue regeneration, enabling the repair and replacement of damaged organs and tissues
-
Medical imaging: Advanced materials like contrast agents and radiotracers are used to enhance the visibility of specific structures or processes in medical imaging techniques, such as magnetic resonance imaging (MRI) and positron emission tomography (PET)
Defense and Security
-
Armor materials: Advanced materials like boron carbide and ultra-high molecular weight polyethylene (UHMWPE) are used to create lightweight and high-strength armor for personal protection and military vehicles
-
Stealth technology: Advanced materials like radar-absorbing coatings and metamaterials are used to reduce the radar cross-section of aircraft and ships, making them less detectable by enemy radar systems
-
Sensors and detectors: Advanced materials like quantum dots and conductive polymers are used to create sensitive and selective sensors for the detection of chemical and biological agents, explosives, and other threats
Challenges and Future Prospects
Despite the significant progress made in the field of advanced materials, there are still several challenges that need to be addressed to fully realize their potential. Some of the key challenges include:
Scalability and Manufacturing
-
Developing cost-effective and scalable manufacturing processes for advanced materials, especially those with complex structures or nanoscale features
-
Ensuring the consistency and reproducibility of material properties across different production batches and facilities
-
Integrating advanced materials into existing manufacturing workflows and supply chains
Sustainability and Environmental Impact
-
Reducing the environmental footprint of advanced material production, including energy consumption, water usage, and greenhouse gas emissions
-
Developing sustainable and eco-friendly production methods, such as using renewable feedstocks and green chemistry principles
-
Addressing the end-of-life management of advanced materials, including recycling, reuse, and safe disposal
Standardization and Regulatory Compliance
-
Establishing standardized testing and characterization methods for advanced materials to ensure consistency and comparability of results across different laboratories and industries
-
Developing regulatory frameworks and safety guidelines for the use of advanced materials in various applications, especially in healthcare and consumer products
-
Harmonizing international standards and regulations to facilitate the global trade and adoption of advanced materials
Despite these challenges, the future prospects for advanced materials are bright. As research and development efforts continue to push the boundaries of material science, we can expect to see new and exciting applications emerge in the coming years. Some of the key trends and opportunities in the field include:
-
Multifunctional materials: Developing materials that can perform multiple functions simultaneously, such as structural support, energy storage, and sensing, enabling the creation of more efficient and compact devices
-
Bioinspired materials: Drawing inspiration from nature to design materials with unique properties and functions, such as self-healing, self-assembly, and adaptive behavior
-
Computational materials design: Using advanced computational methods, such as machine learning and quantum simulations, to accelerate the discovery and optimization of new materials with tailored properties
-
Circular economy: Developing closed-loop systems for the production, use, and recycling of advanced materials, minimizing waste and maximizing resource efficiency
-
Space exploration: Advancing materials for space applications, such as lightweight structures, radiation shielding, and in-situ resource utilization, enabling the exploration and colonization of the Moon, Mars, and beyond
Conclusion
Advanced materials have revolutionized many aspects of modern life, from healthcare and energy to transportation and electronics. By engineering substances with unique properties and functions, we have been able to create materials that can perform tasks that were once thought impossible. As we continue to push the boundaries of material science, we can expect to see even more exciting and transformative applications emerge in the future.
However, the development and deployment of advanced materials also come with significant challenges, including scalability, sustainability, and regulatory compliance. To fully realize the potential of these materials, we need to address these challenges through collaborative research, innovation, and policy-making.
As we look to the future, advanced materials will undoubtedly play a crucial role in solving some of the world's most pressing challenges, from climate change and energy security to healthcare and space exploration. By investing in the development and responsible use of these materials, we can create a more sustainable, resilient, and prosperous future for all.