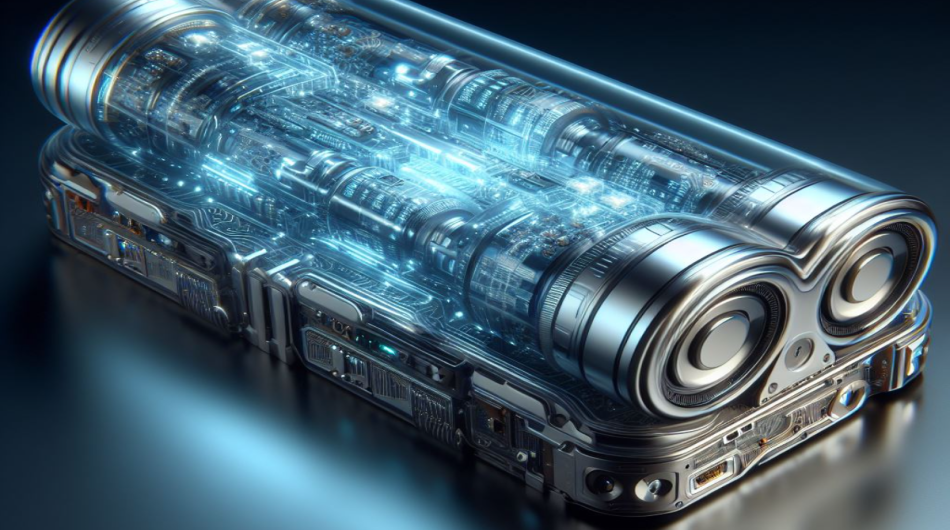
Advanced batteries are a crucial component in the development of clean energy technologies, particularly in the areas of electric vehicles, renewable energy storage, and portable electronics. These batteries offer improved performance, longer lifetimes, and enhanced safety compared to traditional battery technologies. As the world moves towards a more sustainable future, the importance of advanced batteries continues to grow, driving innovation and research in this field. This article provides a comprehensive overview of advanced batteries, including their types, characteristics, applications, challenges, and future prospects.
Types of Advanced Batteries
There are several types of advanced batteries, each with its own unique characteristics and advantages. Some of the most promising and widely researched types include:
Lithium-Ion Batteries (LIBs)
Lithium-ion batteries are currently the most widely used type of advanced battery, powering everything from smartphones to electric vehicles. LIBs offer high energy density, low self-discharge, and long cycle life compared to other battery technologies. They consist of a lithium-based cathode, a graphite anode, and an electrolyte that allows lithium ions to move between the electrodes during charging and discharging.
Cathode Materials
The cathode material is a critical component in determining the performance and cost of LIBs. Some common cathode materials include:
-
Lithium Cobalt Oxide (LCO): Offers high energy density but is relatively expensive and has safety concerns.
-
Lithium Nickel Manganese Cobalt Oxide (NMC): Provides a balance of high energy density, lower cost, and improved safety compared to LCO.
-
Lithium Iron Phosphate (LFP): Known for its excellent safety and long cycle life, but has lower energy density than other cathode materials.
Anode Materials
Graphite is the most common anode material in LIBs due to its low cost and good performance. However, researchers are exploring alternative anode materials to improve the energy density and charging speed of LIBs, such as:
-
Silicon: Offers up to ten times the capacity of graphite but suffers from severe volume expansion during charging, leading to shorter cycle life.
-
Lithium Titanate (LTO): Provides excellent safety and fast charging capabilities but has lower energy density than graphite.
Electrolytes
The electrolyte is responsible for conducting lithium ions between the cathode and anode. Most LIBs use liquid electrolytes consisting of lithium salts dissolved in organic solvents. However, these liquid electrolytes are flammable and can pose safety risks. Researchers are investigating solid-state electrolytes, which offer improved safety and potential for higher energy density.
Lithium-Sulfur Batteries (Li-S)
Lithium-sulfur batteries are an emerging technology that promises higher energy density and lower cost than conventional LIBs. In Li-S batteries, the cathode is composed of sulfur, while the anode is made of lithium metal. During discharge, lithium ions react with sulfur to form lithium polysulfides, which are then converted back to sulfur during charging.
Advantages and Challenges
Li-S batteries offer several advantages, including:
-
High theoretical energy density: Li-S batteries have a theoretical energy density of around 2,600 Wh/kg, compared to 350-400 Wh/kg for current LIBs.
-
Abundant and low-cost materials: Sulfur is an abundant and inexpensive material, potentially reducing the cost of Li-S batteries.
-
Environmental friendliness: Sulfur is non-toxic and environmentally benign, making Li-S batteries a more sustainable option.
However, Li-S batteries also face several challenges that need to be addressed:
-
Polysulfide shuttle effect: During cycling, lithium polysulfides can dissolve in the electrolyte and migrate between the electrodes, causing capacity loss and shorter cycle life.
-
Lithium metal anode: Lithium metal is highly reactive and can form dendrites during charging, leading to short circuits and safety hazards.
-
Low coulombic efficiency: The conversion of sulfur to lithium polysulfides and back is not always complete, resulting in lower coulombic efficiency compared to LIBs.
Researchers are working on various strategies to overcome these challenges, such as developing advanced electrolytes, designing novel cathode structures, and incorporating protective coatings on the lithium metal anode.
Sodium-Ion Batteries (SIBs)
Sodium-ion batteries are another promising alternative to LIBs, particularly for large-scale energy storage applications. SIBs operate on a similar principle to LIBs, with sodium ions moving between the cathode and anode during charging and discharging.
Advantages and Challenges
SIBs offer several advantages over LIBs, including:
-
Abundance and low cost: Sodium is much more abundant and cheaper than lithium, potentially reducing the cost of SIBs.
-
Compatibility with aluminum current collectors: Unlike LIBs, which require expensive copper current collectors, SIBs can use cheaper aluminum current collectors due to the higher reduction potential of sodium.
However, SIBs also face several challenges:
-
Lower energy density: Sodium ions are larger and heavier than lithium ions, resulting in lower energy density compared to LIBs.
-
Limited choice of cathode materials: Many of the high-performance cathode materials used in LIBs, such as NMC and LCO, are not suitable for SIBs due to sodium's larger ionic radius.
-
Anode materials: Graphite, the most common anode material in LIBs, does not work well in SIBs due to the inability of sodium ions to intercalate into its structure. Alternative anode materials, such as hard carbon and tin-based composites, are being explored.
Despite these challenges, SIBs are attracting increasing attention as a complementary technology to LIBs, particularly for applications where cost is more important than energy density, such as grid-scale energy storage.
Redox Flow Batteries (RFBs)
Redox flow batteries are a type of rechargeable battery that stores energy in two separate liquid electrolytes containing dissolved electroactive species. During charging and discharging, these electrolytes are pumped through a cell stack, where the electrochemical reactions occur.
Advantages and Challenges
RFBs offer several unique advantages, including:
-
Decoupled power and energy: The power and energy capacity of RFBs can be independently scaled by adjusting the size of the cell stack and electrolyte tanks, respectively. This allows for greater flexibility in system design and optimization.
-
Long cycle life: RFBs can achieve thousands of charge-discharge cycles without significant degradation, as the electroactive species are not consumed during cycling.
-
Rapid response: RFBs can quickly switch between charging and discharging modes, making them well-suited for applications that require fast response times, such as grid frequency regulation.
However, RFBs also face several challenges:
-
Low energy density: RFBs have lower energy density compared to other battery technologies due to the need for large volumes of liquid electrolytes.
-
Complex system design: RFBs require additional components, such as pumps, pipes, and tanks, which can increase the complexity and cost of the system.
-
Membrane limitations: The performance and lifetime of RFBs are heavily dependent on the properties of the ion-exchange membrane separating the two electrolytes. Developing high-performance, low-cost membranes remains a key challenge.
Despite these challenges, RFBs are gaining traction for large-scale energy storage applications, such as renewable energy integration and grid support services.
Characteristics and Performance Metrics
To evaluate and compare different advanced battery technologies, several key characteristics and performance metrics are used:
Energy Density
Energy density refers to the amount of energy that can be stored in a battery per unit volume (volumetric energy density) or per unit mass (gravimetric energy density). Higher energy density batteries can store more energy in a smaller and lighter package, which is particularly important for applications such as electric vehicles and portable electronics.
Power Density
Power density is a measure of the rate at which a battery can deliver energy per unit volume or mass. Batteries with high power density can provide high currents and fast charging/discharging capabilities, which is crucial for applications that require rapid response times, such as grid frequency regulation and regenerative braking in electric vehicles.
Cycle Life
Cycle life refers to the number of charge-discharge cycles a battery can undergo before its capacity falls below a certain threshold, typically 80% of its initial capacity. A longer cycle life means the battery can be used for a greater number of cycles, reducing the need for frequent replacements.
Coulombic Efficiency
Coulombic efficiency is the ratio of the charge extracted from a battery during discharge to the charge put into the battery during charging. A higher coulombic efficiency indicates that a greater proportion of the stored energy is being utilized, with less energy lost to side reactions or other inefficiencies.
Safety
Battery safety is a critical consideration, particularly for applications where the battery is exposed to extreme temperatures, mechanical stress, or other harsh conditions. Advanced batteries must be designed with robust safety features, such as thermal management systems, protective coatings, and fail-safe mechanisms, to minimize the risk of thermal runaway, fire, or explosion.
Cost
The cost of advanced batteries is a major factor in determining their commercial viability and widespread adoption. Battery costs are typically expressed in terms of dollars per kilowatt-hour ($/kWh) and are influenced by factors such as raw material prices, manufacturing processes, and economies of scale. Reducing battery costs while maintaining or improving performance is a key goal of advanced battery research and development.
Applications of Advanced Batteries
Advanced batteries have a wide range of applications across various sectors, including:
Electric Vehicles (EVs)
Advanced batteries, particularly lithium-ion batteries, are the key enabling technology for electric vehicles. EVs rely on high-energy-density batteries to provide sufficient driving range and power to compete with traditional internal combustion engine vehicles. Advances in battery technology, such as improved cathode and anode materials, are driving down the cost and increasing the performance of EV batteries, making EVs more affordable and accessible to consumers.
Renewable Energy Storage
As the world transitions towards renewable energy sources like wind and solar, energy storage solutions are becoming increasingly important to balance supply and demand and ensure grid stability. Advanced batteries, such as redox flow batteries and sodium-ion batteries, are well-suited for large-scale energy storage applications due to their long cycle life, scalability, and relatively low cost.
Portable Electronics
Advanced batteries have revolutionized the portable electronics industry, enabling the development of smartphones, laptops, and other mobile devices with ever-increasing capabilities and battery life. Lithium-ion batteries, in particular, have become the dominant technology in this sector due to their high energy density, low self-discharge, and long cycle life.
Aerospace and Defense
Advanced batteries are finding applications in the aerospace and defense sectors, where high performance and reliability are paramount. For example, lithium-ion batteries are being used in satellites, drones, and other unmanned aerial vehicles (UAVs) to provide lightweight and high-energy-density power sources. In addition, advanced batteries are being developed for military applications, such as soldier power systems and directed energy weapons.
Medical Devices
Advanced batteries are also playing an increasingly important role in the medical device industry. Implantable medical devices, such as pacemakers and neurostimulators, require small, reliable, and long-lasting power sources. Advanced battery technologies, such as solid-state lithium batteries and thin-film batteries, are being developed to meet the specific requirements of these applications, offering improved safety, biocompatibility, and energy density.
Challenges and Future Prospects
Despite the significant progress made in advanced battery technologies, there are still several challenges that need to be addressed to fully realize their potential. These challenges include:
Raw Material Availability and Sustainability
Many advanced battery technologies rely on rare or expensive raw materials, such as lithium, cobalt, and nickel. As the demand for these materials grows, concerns about their availability, cost, and environmental impact are increasing. Developing sustainable and responsible sourcing strategies, as well as exploring alternative materials and recycling technologies, will be crucial for the long-term viability of advanced batteries.
Manufacturing and Scale-Up
Translating advanced battery technologies from the laboratory to commercial-scale production can be challenging, requiring significant investments in manufacturing infrastructure and process optimization. Scaling up production while maintaining quality, consistency, and safety is a key hurdle that needs to be overcome to reduce costs and enable widespread adoption of advanced batteries.
Safety and Reliability
Ensuring the safety and reliability of advanced batteries is critical, particularly for applications where battery failure could have severe consequences, such as in electric vehicles or aerospace systems. Developing robust safety features, testing protocols, and quality control measures is essential to minimize the risk of battery-related incidents and build public trust in advanced battery technologies.
Recycling and End-of-Life Management
As the use of advanced batteries grows, so does the need for effective recycling and end-of-life management strategies. Recovering valuable materials from spent batteries can help reduce the demand for virgin raw materials, lower costs, and minimize environmental impacts. However, current battery recycling technologies are often complex, expensive, and energy-intensive. Developing more efficient and cost-effective recycling processes, as well as designing batteries with recyclability in mind, will be important for the sustainable development of advanced batteries.
Despite these challenges, the future prospects for advanced batteries are bright. Ongoing research and development efforts are leading to continuous improvements in battery performance, cost, and safety. Some of the key future trends and opportunities in advanced batteries include:
-
Solid-state batteries: Solid-state batteries, which use a solid electrolyte instead of a liquid one, offer the potential for higher energy density, improved safety, and longer cycle life compared to conventional lithium-ion batteries. Several companies and research institutions are actively developing solid-state battery technologies, with some promising early results.
-
Multivalent ion batteries: Batteries that use multivalent ions, such as magnesium or aluminum, instead of lithium ions, could offer higher energy density and lower cost due to the greater abundance of these materials. However, significant challenges, such as the development of suitable cathode materials and electrolytes, need to be overcome to realize the potential of multivalent ion batteries.
-
Advanced manufacturing techniques: Innovations in battery manufacturing, such as 3D printing, roll-to-roll processing, and automated assembly, could help reduce costs, improve quality control, and accelerate the scale-up of advanced battery production.
-
Battery management systems (BMS): Advanced battery management systems, which monitor and control the operation of battery packs, can help optimize performance, extend cycle life, and ensure safety. The development of more sophisticated BMS, leveraging technologies such as machine learning and wireless connectivity, could further enhance the capabilities and reliability of advanced batteries.
-
Second-life applications: As the number of electric vehicles on the road grows, so does the supply of used EV batteries. Finding second-life applications for these batteries, such as in stationary energy storage systems, can help extend their useful life, reduce waste, and create new business opportunities.
Conclusion
Advanced batteries are a critical enabler of the global transition towards clean energy and sustainable transportation. From electric vehicles to renewable energy storage, advanced batteries are powering the technologies that are shaping our future. The development of new battery chemistries, materials, and manufacturing processes is driving continuous improvements in performance, cost, and safety, making advanced batteries increasingly competitive with traditional energy storage solutions.
However, realizing the full potential of advanced batteries will require addressing several key challenges, including raw material availability, manufacturing scale-up, safety and reliability, and end-of-life management. Collaboration between industry, academia, and government will be essential to overcome these challenges and create a sustainable and thriving advanced battery ecosystem.
As research and development efforts continue to push the boundaries of what is possible with advanced batteries, we can expect to see even more transformative applications and opportunities emerge. From powering the next generation of electric vehicles to enabling the widespread adoption of renewable energy, advanced batteries will play a crucial role in building a cleaner, more sustainable, and more resilient future for all.